Sponsored Content by Pion IncReviewed by Maria OsipovaApr 3 2025
In this interview, News Medical talks to Patrick D. Sinko about the role of particle size, dose, and confinement parameters in advancing drug absorption modeling.
Could you explain what a boundary layer is and its significance in particle dissolution?
A boundary layer is formally defined as the region where a property, such as velocity or concentration, reaches 99% of its far-field value. In the case of particle dissolution, we examine the velocity and concentration fields. If we consider a surface with a dissolving particle, the concentration far away from the surface typically approaches zero, which aligns with the classic assumption of bulk concentration being zero.
The particle drift effect can be understood schematically by considering a system with a solid or semi-permeable membrane and a fluid containing particles. We divide this into three regions: Region A, the bulk fluid far from the surface; Region B, the concentration boundary layer; and Region C, which includes the membrane and receiver compartment.
For particle drift to occur, the mass transport should not be rate-limiting, and various factors, such as pH, aqueous solubility, membrane diffusion coefficients, and partition coefficients, can influence this. Because the concentration boundary layer is also linked to the hydrodynamic boundary layer, the fluid dynamics of the system—whether in vitro or in vivo—play a crucial role. In addition, particles must be small enough for the drift effect to activate.
How did you initially approach capturing the particle drift effect in your experiments?
During my PhD, I used a device called the rotating membrane diffusion cell along with a semi-permeable membrane made of polydimethylsiloxane (PDMS), a silicon-based rubber with slight lipophilic properties.
Our data initially showed that the membrane was rate-limiting for transport in most measurements. To address this, I designed a new diffusion cell that could house a much thinner membrane, allowing for our model compound's highest possible absorption rate, ibuprofen.
What technique did you use to create this thinner membrane, and how does it work?
We used spin coating and deposited a water-soluble polymer, polyvinyl alcohol (PVA), onto a large silicon wafer. Since PDMS dissolves in organic solvents while PVA dissolves in water, these layers are orthogonal in solubility.
This allowed us to immerse the wafer in a Petri dish filled with water, where the water infiltrated the radial edge and released the PDMS layer, which was about 10 microns thick. The membrane then self-assembled on the water’s surface, allowing us to extract it and install it into the diffusion cell. Despite being so thin, the material proved to be quite robust.
How did you verify that this new system effectively measured particle dissolution effects?
To ensure our diffusion cell was sensitive enough to detect flux changes due to particle dissolution, we optimized the system by using a small water volume in the upper compartment, which maximized the surface area-to-volume (A/V) ratio.
We then conducted quick solution experiments and found that a fully non-ionized ibuprofen state was necessary to achieve the highest rate-limiting effect of the concentration boundary layer. This refined setup achieved rapid steady-state transport across the membrane with a very short lag time, confirming the improved measurement accuracy.

Image Credit: paulista/Shutterstock.com
What can your model predict in terms of mass transfer and drug flux?
Our model incorporates modern mass transfer theories, including recent work by Dr. James Brasseur and his former postdoc Yang Shi Wang. One key enhancement we observe is related to the Sherwood number, which influences mass transfer efficiency.
As the particle radius decreases, the flux increases as expected in the particle drift phenomenon. However, there is also a clear dose dependence, meaning the effect varies with suspension concentration.
How does your model compare to traditional particle dissolution models?
The traditional film model for particle dissolution assumes that flux behavior depends primarily on particle size relative to the concentration boundary layer. In this model, large particles show a dose-dependent increase in flux, while very small particles quickly reach their maximum flux regardless of dose.
When we account for particle dissolution within the boundary layer, our model predicts that small particles can achieve significantly higher flux than previously expected. This is an exciting result. This was confirmed by experimental data, as seen in Sinko PD et al. 2023 Molecular Pharmaceutics 21 (1), 201-215
Can you explain how you tested the model using different particle fractions of ibuprofen and what insights you gained from the dissolution permeation experiments?
To test the model fully, we used three different particle fractions of ibuprofen. First, we had the finest material, which was high shear wet sieved. This was our positive case, where all the particles were smaller than the concentration boundary layer.
Then, we tested very large dry-sieved particles, which acted as a pure negative case since they were all larger than the boundary layer length scale. Finally, to challenge the model further, we created a material containing smaller and larger particles relative to the boundary layer scale.
We observed some key trends in our dissolution permeation experiments using a new diffusion cell. We used two different diffusion cells to introduce a slight variation in hydrodynamics, with the concentration boundary layer being about 10 microns thicker in one of them. This allowed us to test the sensitivity of the model. The results showed smaller particles had a higher total permeated mass over time and exhibited steeper slopes in concentration profiles.
The finer particles dissolved and permeated more efficiently through the PDMS membrane, while the larger particles had a much lower flux. We observed that the flux across the membrane increased as the dose number increased. These findings help us better understand how particle size influences permeation and dissolution dynamics in our system.
How well did the model predictions align with the experimental data for different particle sizes?
When we tested particles smaller than the concentration boundary layer thickness, our model showed strong agreement with the experimental data. There was a slight difference in lower dose numbers due to hydrodynamic effects, but this difference disappeared as the dose number increased, approaching 1000.
For the pure negative case, where all particles were larger than the boundary layer thickness, we again saw good agreement between the model’s solution predictions and the data from the diffusion cell. However, the data seemed to align more closely with traditional film model assumptions rather than the underprediction suggested by our model. This is expected because our model is not designed to predict particle dissolution within the boundary layer when the particles are too large to exist within it.
We observed slightly higher fluxes than predicted by the traditional film model. Given that we were working with a very shallow water column, this is likely due to some settling effects. Even at high agitation speeds, it is probable that some of the larger particles settled, influencing the observed flux values.
How did the in-between particle fraction behave in the dissolution permeation experiments?
We observed an interesting trend for the in-between particle fraction, which contained smaller and larger particles relative to the concentration boundary layer thickness. When using the mass-average radius, the behavior at lower dose numbers was more in line with traditional film theory assumptions.
However, as the dose number increased, the data deviated significantly from those predictions. This suggested that something beyond simple film theory influenced the dissolution and permeation process at higher dose numbers.
What role did particle size distribution play in dissolution and permeation, and how did using the number-average radius impact the model’s predictions?
To understand this behavior, we considered the particle drift effect, where smaller particles infiltrate the concentration boundary layer, increasing flux across the membrane. Assuming good mixing, a higher proportion of small particles exist within the boundary layer, significantly affecting dissolution and permeation rates.
Since smaller particles dissolve faster due to their larger surface area-to-volume ratio, they contribute more to the overall process.
When we analyzed the number-average radius instead of the mass-average radius, our model’s predictions closely matched the experimental data. This indicated that, at steady state, the smaller particles dominated dissolution and permeation.
Even though mass and volume fractions suggested that most particles The number fraction distribution was larger than the boundary layer, showing that a significant portion, or even the majority, were microparticles capable of dissolving within the boundary layer.
Can you explain the design evolution of the diffusion cell and how it impacted the experimental setup?
During the development of the diffusion cell, we initially used sonic sieve meshes to support the polymer membrane before transitioning to a laser-cut stainless steel support system.
The final design featured a precisely cut grid pattern that provided a sturdy and uniform surface for the thin membrane. However, before we had access to laser cutting, we had to cut and shape sonic sieve meshes to fit inside the glass chamber.
The problem with the mesh system was that its woven wires created pockets nearly a millimeter deep, unlike the flat planar surface of the laser-cut support. This affected particle behavior significantly—while the final diffusion cell allowed smooth fluid flow across the membrane, the mesh system trapped particles in these wells, altering how they interacted with the boundary layer.
What unexpected effects did the mesh-based support system introduce, and how did they relate to biological systems?
One surprising effect of the mesh system was that trapped particles exhibited drift-like behavior. Because they were pinned in place within these deep wells, they created localized mass sources, increasing measured flux. However, since the particles were not freely moving or convecting with the solution, we lost the usual dependency on particle radius.
This observation led us to an interesting biological parallel. In the human body, structures like microvilli and villi contain deep crypts that can concentrate particles, enhancing nutrients and pharmaceutical absorption. The way particles accumulated in the mesh wells mimicked how the body might use these structures to optimize absorption efficiency, reinforcing that microenvironments can significantly influence transport and uptake mechanisms.
Pion Inc. provides an excellent platform for sharing cutting-edge research and fostering scientific discussions. It was a pleasure to present my work in collaboration with Pion, and I appreciate their commitment to advancing pharmaceutical science through knowledge exchange.
Watch Free Webinar: Exploring drug absorption models through particle size, dose, and confinement
About Patrick D. Sinko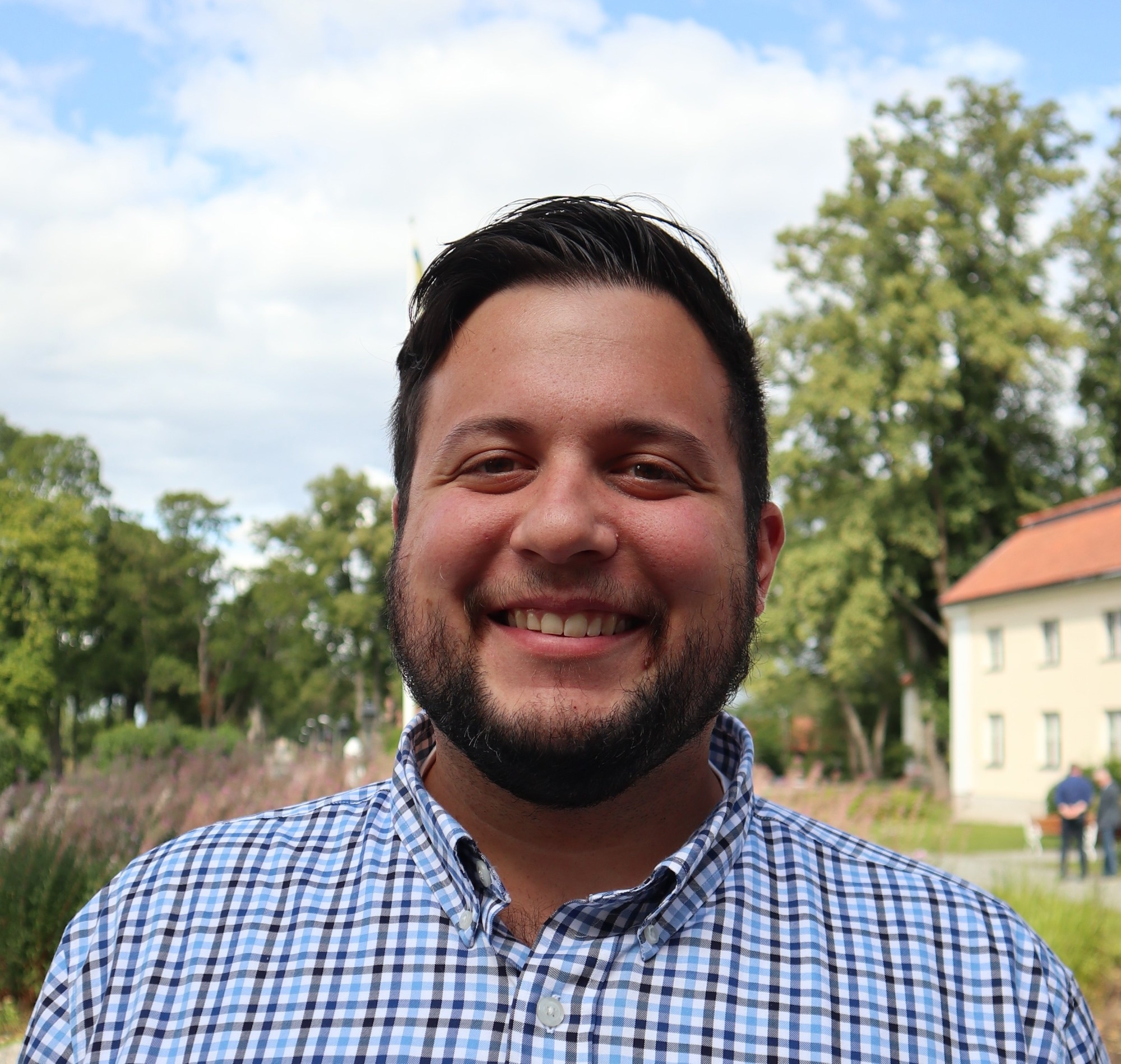
Patrick D. Sinko is a researcher focused on reducing and replacing animals during preclinical drug development. He obtained his bachelor's of science in Materials Science and Engineering at Virginia Tech in 2013, his master's in Materials Science and Engineering at the University of Michigan in 2018, and his PhD in Pharmaceutical Sciences in 2019 at the University of Michigan.
During his PhD, he developed a methodology to produce ultra-thin polymer membranes that could be used to study simultaneous dissolution-permeation processes. After moving to the Molecular Pharmaceutics group at Uppsala University, he continues to work on designing a biorelevant benchtop device that will examine the dissolution and absorption processes of advanced drug delivery systems.
About Pion Inc
When data matters we apply out of the box problem solving abilities to help you reach a confident conclusion on your drug characterization challenges.
Pion supports the development of lifesaving and life-enhancing drugs by providing tools for drug developers, formulations scientists, and pharmaceutical production. For early-stage drug developers, our cutting-edge analytical technologies and services enable in vitro measurements that provide essential data to improve candidate selection and formulations decisions. Later in development, high-pressure homogenizers enable particle size reduction and ensure material consistency from bench- to production-scale.