Sponsored Content by MerckReviewed by Emily MageeJan 15 2024
Organ failure impacts millions of patients each year and costs hundreds of billions of US Dollars. Over the last 30 years, scientists have utilized a combination of tools, methods, and molecules of biology, chemistry, physics, and engineering to fabricate new tissues to restore or replace functional organs.1
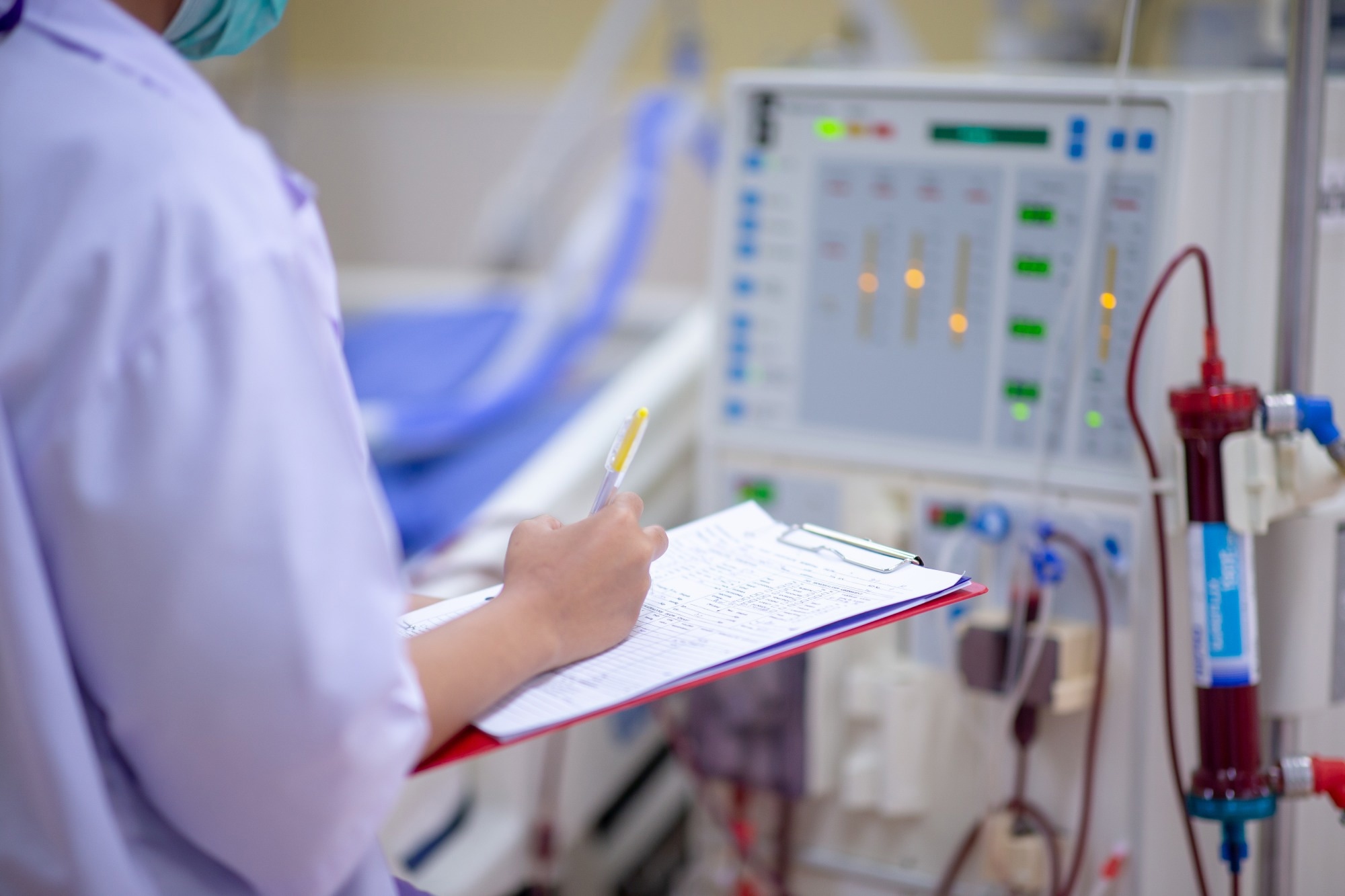
Image Credit: Hospital man/Shutterstock.com
This field is referred to as tissue engineering and has three main subfields, as displayed in Figure 1:
- The ex vivo generation of implantable tissue with material scaffolds involving cells and growth factors;
- Biohybrid materials with or without cells to stimulate in vivo regeneration;
- Models of ex vivo tissue to examine tissue formation and pathological processes in combination with drugs.
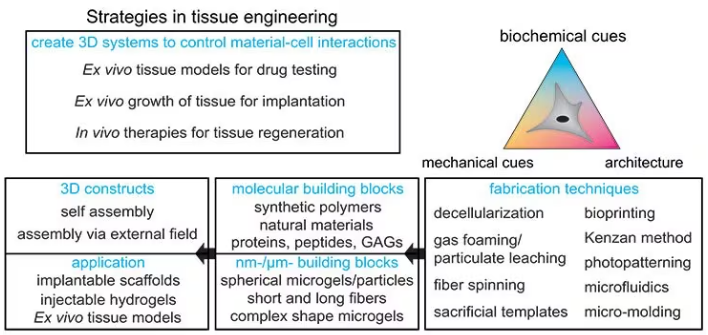
Figure 1. Strategies for designing tissue engineering constructs: Different fabrication techniques can be used to combine molecular and nanomicrometer size building blocks to form 3D constructs for implantable scaffolds, injectable applications, or ex vivo tissue models. Image Credit: Merck
Material selection for tissue constructs
Over recent decades, scientists have developed two key paths for the fabrication of tissue constructs in various sizes and complexities, namely injectable hydrogels and implantable scaffolds.
Scaffolds for ex vivo tissue models or implantation can be constructed with intricate architectures because the materials are processed outside the body. One method is the decellularization of natural tissues to product structures that are highly like the original, as shown in Figure 2A.
The first animal experiment was conducted in 1995, with decellularized small intestinal submucosa from pigs being implanted into dogs to provide better Achille’s tendon repair. A decellularized trachea was successfully implanted in a 10-year-old in 2010.
In initial experiments, tissues were decellularized by immersing the tissue in a detergent solution. However, more recent techniques utilize the native vascular network for perfusion and recellularization,2 facilitating the creation of whole liver and other types of grafts.3
Despite decellularization methods having significantly improved to maintain most of the essential extracellular matrix (ECM) components, there are still many challenges involved.
These advantages encompass inflammatory responses, improved preservation of the biochemical and physical integrity of complete ECMs, and the enhancement of bioreactors for efficient recellularization.
To systematically investigate interactions between materials and cells, researchers are constructing constructs using both synthetic and natural materials, which are two components with distinct characteristics.
Although natural materials, including fibrin, collagen, and Matrigel® contain many biological signals, artificial synthetic ECMs (referred to as aECM) are prepared using a small number of well-defined building blocks.
To create functional tissue, these matrices simulate the biochemical, mechanical, and structural properties of the ECM, such as degradability. The biocompatible chemistry and physiological conditions must be preserved to incorporate live cells during preparation.
At present, most materials are biohybrid systems that have the controllability of synthetic materials as well as the biological activity of natural compounds.
Fabrication methods for solid implantable scaffolds
Material properties must be designed at multiple scales, from the chemical, physical, and biochemical properties of the molecules to the nano- and micrometer scale of the structural elements and porosity to the macroscopic architecture.
One of the first techniques employed for the preparation of implantable scaffolds was solvent casting, which involves a polymer being dissolved in an evaporating organic solvent with leachable porogens, e.g., salt particles.4
However, the cytotoxicity of organic solvents makes it impossible to add proteins or cells. As a result, more biocompatible techniques, such as gas-foaming particulate leaching and freeze-drying, have been developed that allow the incorporation of bioactive molecules as well as the creation of more complex architectures.
For instance, aligned channels may be fabricated to simulate oriented tissues like the spinal cord, as shown in Figure 2B. Another technique is fiber spinning, which creates fibrous mats with random or oriented fibers in which the fiber density, diameter, and topography can be controlled.
Electrospinning is the most commonly used spinning method and involves electrostatic forces inducing a Taylor cone to accelerate the solution towards a collector with the opposite polarity. As the solvent evaporates, this produces synthetic fibers, as displayed in Figure 2C.5
In 1978, the first electrospun mats for tissue engineering were utilized as vascular prostheses.
Solvent-assisted spinning is another method that does not apply an electric field but involves the fibers being mechanically pulled and collected by a rotating drum. This allows fibers with larger diameters to form, as well as more controllable fiber orientation, inter-fiber distances, and fiber topography.
Wet spinning involves polymers being dissolved in a non-volatile solvent and subsequently extruded in a solution. This washes the solvent out, rapidly producing fibers.
Thermostable polymers may be molten (without a solvent) and pressed out of a nozzle during melt-spinning, then cooled by airflow between the collector and the nozzle. This method does not require further washing steps.
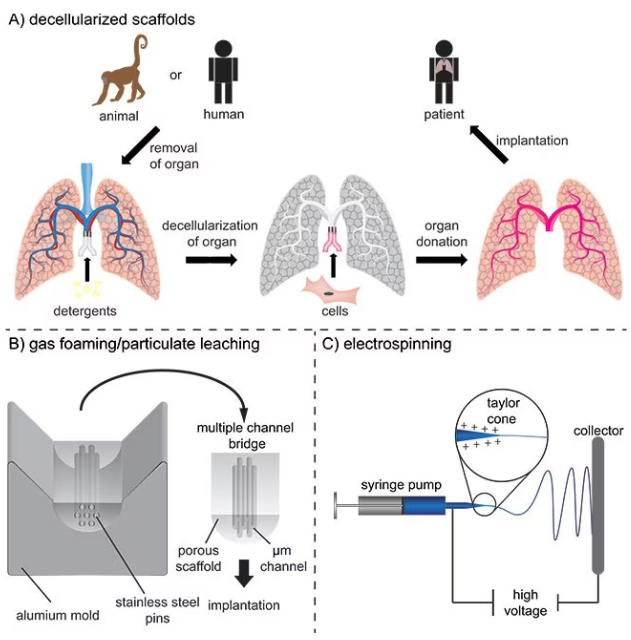
Figure 2. A) Decellularization of tissues and subsequently recellularization for the production of vascularized tissue engineering constructs for potential implantation. B) Production of multiple channel spinal cord bridges using a gas foaming/particulate leaching technique. C) Electrospinning of nano- and micrometer-sized fibers for implantable scaffolds. Image Credit: Merck
Fabrication methods for hydrogel-based scaffolds
The above-mentioned fabrication techniques produce solid implantable scaffolds, but natural tissues are typically softer and viscoelastic. Hydrogels are water-containing networks that simulate these properties and are prepared by crosslinking hydrophilic synthetic or natural polymers, proteins, or sugars.
Crosslinks are created via physical interactions and reversible bonds and/or by covalent bonds and chemical reactions. For instance, bonds in gelation may be stimulated by pH, enzymes, ionic interactions, or temperature.6
Synthetic polymers, glycosaminoglycans (GAGs), and recombinant proteins are employed for the preparation of hydrogels to reduce batch-to-batch variability and prevent potential pathogenic contaminations.
Building blocks, including poly(ethylene glycol) (PEG) or polyacrylamide, ECM fragments, bioactive peptides, and proteins, are mixed within the network to generate synthetic hydrogels.
The linkers between the molecules or the molecules themselves may be designed with ester bonds amenable to hydrolysis or matrix metalloproteinase (MMP) cleavage sites if degradation is desired.
By integrating knowledge of biocompatible chemical reactions, biochemistry, and physical chemistry, a diverse range of hydrogels with varying mechanical and biological attributes has been developed to cater to a wide array of applications.
The remaining challenge is decoupling the effects of different parameters on cell behavior, including stiffness, mesh size, ligand spacing, degradation rate, and topography.
Another challenge is that most conventional hydrogels have nano-size pores, which inhibit cell migration when covalent and nondegradable crosslinks restrict efficient nutrient perfusion.
When cells are mixed inside the hydrogels, either reversible crosslinks or matrix degradation are necessary to allow the cells to spread and migrate. As a result, hydrogels with dynamic bonds have recently been created and examined to better simulate the strain-stiffening, viscoelastic, and fibrous properties of the native ECM.
Macroporous hydrogels have been created to further enhance the infiltration of endogenous cells in vivo, which is complex due to cells choosing the least resistant path around the hydrogel.
Here, strategies vary for implants and injectable materials. For implants, sacrificial porogens or templates can produce macroscopic pores and hydrogels with shape-recovering complex structures, as displayed in Figure 3A,7 but may not enable simultaneous cell encapsulation into the hydrogel.
To overcome this challenge, bioprinting has emerged as a viable method for printing 3D hydrogel constructs in combination with proteins and cells. There are three bioprinting methods, namely micro-extrusion, inkjet printing, and laser-assisted printing, as presented in Figure 3 B–D.8
Although inkjet printing is an accessible and cost-effective technique that deposits the material droplet-wise, micro-extrusion prints continuous lines and patterns, allowing for higher viscosities and cell densities. However, extrusion is a slower process, and high shear stresses make the maintenance of cell viability challenging.
Laser-assisted bioprinting involves a focused, pulsed laser shooting micro-beats from a ribbon onto specific positions on a receiving substrate. This method is more elaborate, complex, and expensive and demands fine-tuning for each cell type and application.
However, laser-assisted bioprinting can be used over a wide range of viscosities and high cell densities and allows cells to remain viable during the process.
While these printing methods have advanced significantly, a new generation of bioinks is required to simulate the ECM properties without compromising printability in the presence of cells.
The ideal bioink is a non-cytotoxic liquid that crosslinks only on demand (to avoid nozzle clogging), prevents the cells from being subjected to mechanical and thermal stress, and allows high cell densities.
The crosslinking initiator should not harm the cells, and the crosslinking process must be both manageable and fast enough to attain high precision. The resultant hydrogel should exhibit cell-adhesive properties, be degradable, and offer adjustable stiffness.
As an alternative to bioink, the Kenzan method directly prints cell spheroids without relying on a supportive hydrogel scaffold (refer to Figure 3E). In this approach, cell spheroids are strategically positioned on micro-needles to create a desired structure, such as vascular tubes formed by the fusion of these spheroids.9
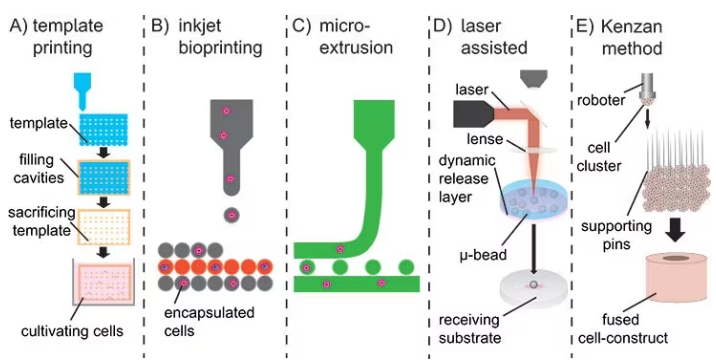
Figure 3. A) Sacrificial templates to create macroscopic hydrogels. B) Droplet-wise inkjet bioprinting. C) Continuous line printing using micro-extrusion. D) Laser-assisted bioprinting using a focused pulsed laser to shoot micro-beats on a receiving substrate. E) Kenzan method to print cell spheroids directly without using supporting hydrogel scaffolds. Image Credit: Merck
Hydrogel precursor solutions may be injected as a liquid and subsequently crosslinked in situ under physiological conditions employing a minimally invasive procedure.
This enables adaptation to irregular shapes inside the body and forms a close interface with surrounding tissue, provided that the crosslinking mechanism is fully optimized — fast enough to avoid leakage but slow enough to allow handling and precise injection.
This method has two notable limitations: firstly, the absence of macroscopic pores hinders the infiltration of endogenous cells, and secondly, it does not facilitate the formation of hierarchical and oriented structures necessary for guiding cell growth.
One approach to addressing the lack of macroscopic pores is the injection of a pre-crosslinked microgel that contains some amount of remaining reactive groups. Following this, the microgels are linked together through a secondary crosslinking mechanism.
Macroscopic pores of different sizes can be produced based on the diameter of the spherical microgels.
These structures are called interconnected microporous annealed particle scaffolds (MAPs), and they considerably improve cell infiltration and tissue healing relative to conventional hydrogels with the same polymer composition, as shown in Figure 4A.10
Microgels for this purpose are prepared using microfluidics with different materials, such as alginate or PEG, and covalently linked together either via enzymatic reactions or specific interactions.11
Utilizing guest-host chemistry, microgels are crosslinked to obtain shear-thinning properties inside these MAPs. This enables this material to be used as a bioink.12
An alternative method for obtaining larger pores is the production of ECM-like fibrous structures through the self-assembly of highly defined molecules using supramolecular chemistry.
The pore size and strain-stiffening properties of the structure differ depending on the lengths and properties of the molecular building blocks, as displayed in Figure 4B.13
These microporous structures provide the benefit of cells interacting with each other instead of with the ECM. These cell-cell interactions are vital for many biochemical processes, such as cell organization and maturation.
For instance, the stemness of neural progenitor cells in the absence of differentiation factors is heavily reliant on the degradation rate and mechanism of the hydrogel. As a result, it is also reliant on the capability of the cells to remodel the matrix over time and exert cadherin-mediated cell-cell interactions.14
Additionally, during the development of the mesenchyme, cells initially demonstrate significant interactions with the ECM, but as time passes, they begin to interact more with each other via cell-cell interactions. This is a process that is not yet fully understood.15
The second issue is the frequently isotropic character of injectable hydrogels. To induce orientation and hierarchy into these hydrogels, various technologies are in development, such as oriented self-assembling nanofibers, photopatterning, and the formation of guiding structures using an external magnetic field.
Photo-patterning employs light to produce high-resolution structures of biochemical and/or mechanical patterns inside a hydrogel that function as guiding cues.
In this method, photosensitive molecules are activated with a focused laser beam, leading to local crosslinking and subsequent degradation, stiffening, exposure of functional groups, or post-modifications (as shown in Figure 5A).16
Two-photon lithography can attain superior resolution in the z-direction. However, aside from laser ablation, it is currently limited to the photopatterning of relatively thin hydrogel layers, typically measuring only a few millimeters in thickness. Consequently, this method is currently unsuitable for fabricating larger tissues such as the heart or liver.
In the case of self-assembly, peptide amphiphiles transform into nanofibers with a high aspect ratio. These nanofibers can be aligned over a range of up to one centimeter by manually dragging the fibrous structure into a saline solution (refer to Figure 5B).
These supramolecular filaments can be functionalized with bioactive peptides and support aligned neuronal cell growth.17
Despite this method enabling a minimally invasive in vivo application, variation of the nanofiber dimensions is limited, and the alignment of the filaments is dependent on the flow direction inside the needle.
Alternatively, magnetic fields can be employed to create chains of magnetic particles within hydrogels (refer to Figure 5C). These strings act as guiding elements to orient cells, but controlling their dimensions and shapes is challenging, and a high concentration of cytotoxic iron oxide particles is also required.
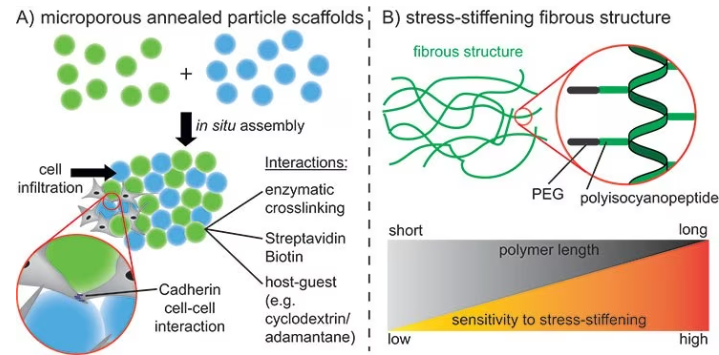
Figure 4. A) Assembly of spherical microgels using different interactions to promote cell infiltration into the scaffold and cell-cell interactions. B) Stressstiffening fibrous structures using polyisocyanopeptides. Different polymer lengths can be used to control the stress-stiffening properties. Image Credit: Merck
To enhance control over the anisotropy of injectable hydrogels while minimizing the use of iron oxide, the concept of Anisogel (Anisotropic hydrogel) was conceived. Anisogel is a hybrid hydrogel that comprises two components: a precursor solution and magneto-responsive, micron-scale, rod-shaped guiding elements.
Following injection, the guiding elements align in the presence of an external magnetic field in the millitesla range within one minute. Meanwhile, the surrounding precursor solution undergoes crosslinking to secure the alignment of these oriented elements in approximately 2–3 minutes.
The elements can be rod-shaped microgels created by either in-mold polymerization or microfluidics or short polymeric fibers that are fabricated via a spinning or microcutting combinatorial technique, as shown in Figure 5 D–F.
The magneto-responsiveness is realized by including small amounts of superparamagnetic iron oxide nanoparticles (SPIONs) inside the micro-objects during fabrication.
This technology enables a high degree of control over parameters like bioactivity, stiffness, dimensions, topography, and concentration of the guiding micro-elements, as well as the bioactivity and stiffness of the surrounding hydrogel (Figure 5G).18
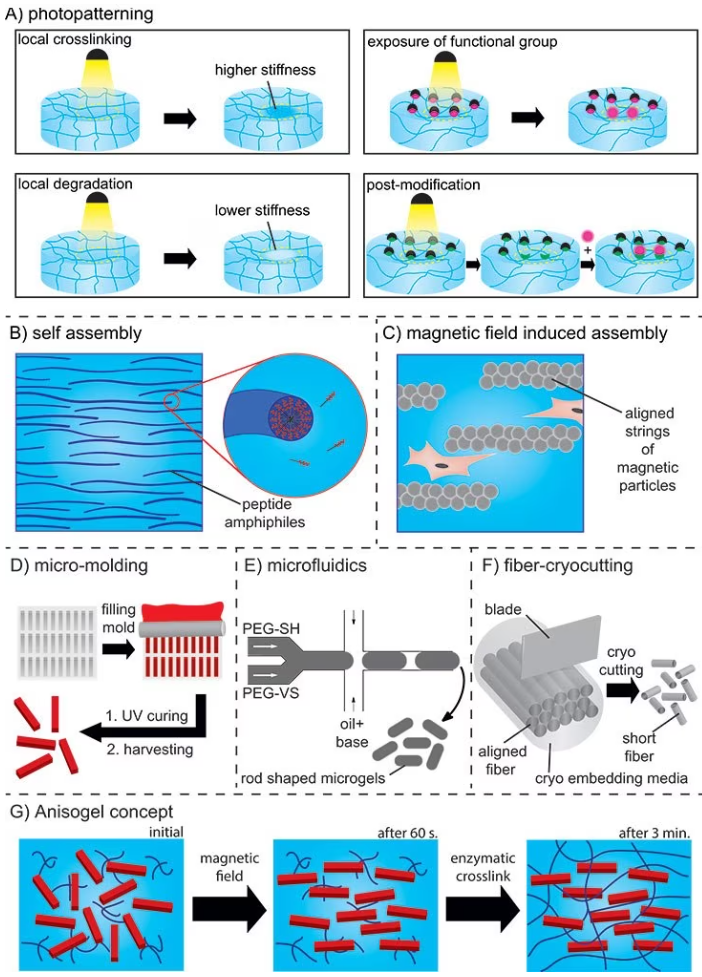
Figure 5. A) Photopatterning resulting in local crosslinking or degradation, exposure of functional groups, or post-functionalization. B) Self-assembly of peptide amphiphiles. C) Aligned strings of magnetic particles in a magnetic field. D) In mold polymerization method and E) Microfluidics for preparation of rod-shaped microgels (PEG-SH: PEG-thiol and PEG-VS: PEG-vinylsulfone). F) Spinning/microcutting combinatorial method for short fiber production. G) Concept of the Anisogel. Image Credit: Merck
Summary and outlook
The majority of the methods described in this article are still under development and optimization.
However, they are also being employed to identify the factors that control cell behavior and tissue formation, as well as for turning cells or biopsy samples into organoids to examine the creation of mini-tissues, the onset of pathologies, and the impact of certain drugs, as displayed in Figure 6.
While Matrigel® is the most efficient hydrogel material for producing organoids, it is not well characterized. This makes a reductionist approach infeasible.19 As a result, synthetic approaches are being investigated to control and guide cell behavior in a more reproducible and robust way.20
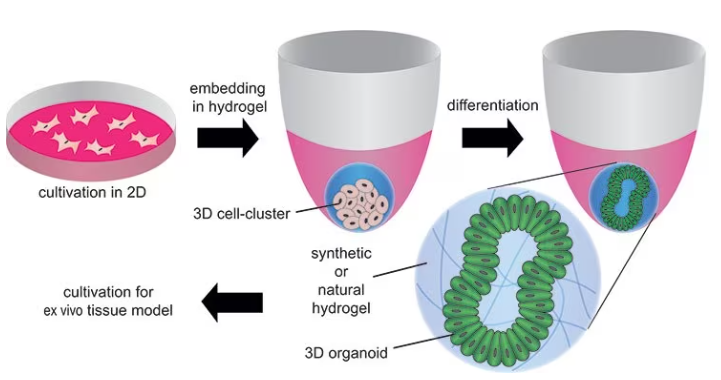
Figure 6. Formation of organoids by embedding stem cells in synthetic or natural hydrogels and differentiating/maturating them by using differentiation factors. Image Credit: Merck
Although organoids are already a valuable tool for ex vivo tissue models, they are on the millimeter scale, which limits their use as implantable tissue engineering constructs for replacing damaged tissue.
However, as more is understood regarding tissue formation and the role of cell-ECM and cell-cell interactions during stem cell differentiation and maturation, these methods show great potential for creating complex tissue constructs via natural organizational processes without each cell being individually guided.
By identifying the minimal amount of “external” triggers necessary within an artificial environment, it may be possible to focus on and activate natural healing and regeneration processes. This will be a crucial aspect of producing complete organs in the future.
Acknowledgments
Produced from materials originally authored by David B. Gehlen and Laura De Laporte at DWI, Leibniz-Institute for Interactive Materials, Aachen, Germany.
References
- Place ES, Evans ND, Stevens MM. 2009. Complexity in biomaterials for tissue engineering. Nature Mater. 8(6):457-470. https://doi.org/10.1038/nmat2441
- Ott HC, Matthiesen TS, Goh S, Black LD, Kren SM, Netoff TI, Taylor DA. 2008. Perfusion-decellularized matrix: using nature’s platform to engineer a bioartificial heart. Nat Med. 14(2):213-221. https://doi.org/10.1038/nm1684
- Uygun BE, Soto-Gutierrez A, Yagi H, Izamis M, Guzzardi MA, Shulman C, Milwid J, Kobayashi N, Tilles A, Berthiaume F, et al. 2010. Organ reengineering through development of a transplantable recellularized liver graft using decellularized liver matrix. Nat Med. 16(7):814-820. https://doi.org/10.1038/nm.2170
- Choudhury M, Mohanty S, Nayak S. 2015. Effect of Different Solvents in Solvent Casting of Porous PLA Scaffolds?In Biomedical and Tissue Engineering Applications. j biomater tissue eng. 5(1):1-9. https://doi.org/10.1166/jbt.2015.1243
- Sill TJ, von Recum HA. 2008. Electrospinning: Applications in drug delivery and tissue engineering. Biomaterials. 29(13):1989-2006. https://doi.org/10.1016/j.biomaterials.2008.01.011
- Rose JC, De Laporte L. 2018. Regenerative Medicine: Hierarchical Design of Tissue Regenerative Constructs (Adv. Healthcare Mater. 6/2018). Adv. Healthcare Mater.. 7(6):1870026. https://doi.org/10.1002/adhm.201870026
- Torres?Rendon JG, Femmer T, De Laporte L, Tigges T, Rahimi K, Gremse F, Zafarnia S, Lederle W, Ifuku S, Wessling M, et al. 2015. Bioactive Gyroid Scaffolds Formed by Sacrificial Templating of Nanocellulose and Nanochitin Hydrogels as Instructive Platforms for Biomimetic Tissue Engineering. Adv. Mater.. 27(19):2989-2995. https://doi.org/10.1002/adma.201405873
- Murphy SV, Atala A. 2014. 3D bioprinting of tissues and organs. Nat Biotechnol. 32(8):773-785. https://doi.org/10.1038/nbt.2958
- Moldovan NI, Hibino N, Nakayama K. 2017. Principles of the Kenzan Method for Robotic Cell Spheroid-Based Three-Dimensional Bioprinting. Tissue Engineering Part B: Reviews. 23(3):237-244. https://doi.org/10.1089/ten.teb.2016.0322
- Griffin DR, Weaver WM, Scumpia PO, Di Carlo D, Segura T. 2015. Accelerated wound healing by injectable microporous gel scaffolds assembled from annealed building blocks. Nature Mater. 14(7):737-744. https://doi.org/10.1038/nmat4294
- Hu Y, Mao AS, Desai RM, Wang H, Weitz DA, Mooney DJ. Controlled self-assembly of alginate microgels by rapidly binding molecule pairs. Lab Chip. 17(14):2481-2490. https://doi.org/10.1039/c7lc00500h
- Mealy JE, Chung JJ, Jeong H, Issadore D, Lee D, Atluri P, Burdick JA. 2018. Injectable Granular Hydrogels with Multifunctional Properties for Biomedical Applications. Adv. Mater.. 30(20):1705912. https://doi.org/10.1002/adma.201705912
- Kouwer PHJ, Koepf M, Le Sage VAA, Jaspers M, van Buul AM, Eksteen-Akeroyd ZH, Woltinge T, Schwartz E, Kitto HJ, Hoogenboom R, et al. 2013. Responsive biomimetic networks from polyisocyanopeptide hydrogels. Nature. 493(7434):651-655. https://doi.org/10.1038/nature11839
- Madl CM, LeSavage BL, Dewi RE, Dinh CB, Stowers RS, Khariton M, Lampe KJ, Nguyen D, Chaudhuri O, Enejder A, et al. 2017. Maintenance of neural progenitor cell stemness in 3D hydrogels requires matrix remodelling. Nature Mater. 16(12):1233-1242. https://doi.org/10.1038/nmat5020
- Cosgrove BD, Mui KL, Driscoll TP, Caliari SR, Mehta KD, Assoian RK, Burdick JA, Mauck RL. 2016. N-cadherin adhesive interactions modulate matrix mechanosensing and fate commitment of mesenchymal stem cells. Nature Mater. 15(12):1297-1306. https://doi.org/10.1038/nmat4725
- Kloxin AM, Kasko AM, Salinas CN, Anseth KS. 2009. Photodegradable Hydrogels for Dynamic Tuning of Physical and Chemical Properties. Science. 324(5923):59-63. https://doi.org/10.1126/science.1169494
- Zhang S, Greenfield MA, Mata A, Palmer LC, Bitton R, Mantei JR, Aparicio C, de la Cruz MO, Stupp SI. 2010. A self-assembly pathway to aligned monodomain gels. Nature Mater. 9(7):594-601. https://doi.org/10.1038/nmat2778
- Rose JC, Cámara-Torres M, Rahimi K, Köhler J, Möller M, De Laporte L. 2017. Nerve Cells Decide to Orient inside an Injectable Hydrogel with Minimal Structural Guidance. Nano Lett.. 17(6):3782-3791. https://doi.org/10.1021/acs.nanolett.7b01123
- Dutta D, Heo I, Clevers H. 2017. Disease Modeling in Stem Cell-Derived 3D Organoid Systems. Trends in Molecular Medicine. 23(5):393-410. https://doi.org/10.1016/j.molmed.2017.02.007
- Gjorevski N, Sachs N, Manfrin A, Giger S, Bragina ME, Ordóñez-Morán P, Clevers H, Lutolf MP. 2016. Designer matrices for intestinal stem cell and organoid culture. Nature. 539(7630):560-564. https://doi.org/10.1038/nature20168
About Merck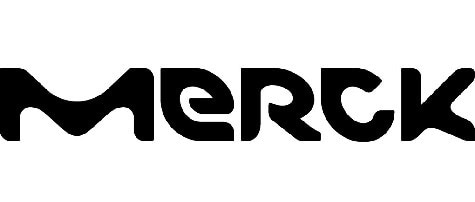
Our pursuit is progress for people everywhere. That's why we take a closer look at things, ask questions, and think ahead.
We've been around for more than 350 years, yet our majority owners are still the descendants of Friedrich Jacob Merck, the man who founded our company in Darmstadt, Germany in 1668.
From advancing gene-editing technologies and discovering unique ways to treat the most challenging diseases to enabling the intelligence of devices – the company is everywhere.
We are Merck. The only exceptions are the United States and Canada. Here we operate as EMD Serono in the Biopharma business, as MilliporeSigma in the Life Science business, and as EMD Performance Materials in the materials business.
Our life science business
We provide infinite solutions to solve the toughest problems in life science in collaboration with the global scientific community. Our tools, services, and digital platforms empower scientists and engineers at every stage, helping deliver breakthrough therapies more quickly.
Focus areas
With our three business units, we are a leading worldwide supplier of tools, high-grade chemicals, and equipment for academic labs, biotech, and biopharmaceutical manufacturers, as well as the industrial sector.
- Research Solutions provides our academic customers with the chemicals and tools needed to make scientific discovery easier and faster.
- Process Solutions provides drug manufacturers with process development expertise and technologies, such as continuous bioprocessing.
- Applied Solutions offers both testing kits and services to ensure that our food is safe to eat and our water is clean to drink.
Sponsored Content Policy: News-Medical.net publishes articles and related content that may be derived from sources where we have existing commercial relationships, provided such content adds value to the core editorial ethos of News-Medical.Net which is to educate and inform site visitors interested in medical research, science, medical devices and treatments.