Sponsored Content by MerckReviewed by Emily MageeFeb 1 2024
In the last two decades, tissue engineering and regenerative medicine have emerged as vital interdisciplinary fields spanning biology, chemistry, engineering, and medicine.1,2
Image Credit: Choksawatdikorn/Shutterstock.com
These fields aim to facilitate healing and restore lost function in damaged or diseased tissues and organs by integrating scaffolds, cells, and biological signaling molecules. This combination aims to recreate functional biological substitutes, imitating native tissues and their functions.3
While one goal is to produce viable tissues and organs for transplantation, success in human patients has been limited, except for thin skin and avascular cartilage,4 mainly due to the intricate nature of tissue biology.
The conventional approach in tissue engineering involves seeding cells onto a solid, porous biomaterial known as a scaffold, with or without growth factors. This technique aims to encourage cells to develop into desired tissues, replicating the complexity found in natural biological structures.5
However, achieving the desired result is rare, as the mixture of the three components – cells, growth factors, and biomaterials – typically does not effectively facilitate the formation of a well-defined spatial distribution at the microscale level, which is essential for creating tissue-like structures.
Three-dimensional (3D) printing, or additive manufacturing (AM), presents a promising solution in tissue engineering. Due to its layer-by-layer process, 3D printing allows the creation of intricate geometries using multiple materials (Figure 1).
The evolution of 3D printing for tissue engineering has given rise to a new technology known as bioprinting, defined as "the use of material transfer processes for patterning and assembling biologically relevant materials, molecules, cells, tissues, and biodegradable biomaterials with a prescribed organization to achieve one or more biological functions."6
Bioprinting offers the potential for personalized and precision medicine by crafting anatomically shaped implants with tissue-like complexity, utilizing a patient's own cells. Presently, 3D bioprinting technologies can be categorized into two main groups: acellular and cellular constructs.7
Acellular bioprinting is used to manufacture the scaffold and biomaterial itself in the absence of cells during the printing process. This method provides increased precision and greater shape complexity compared to cellular constructs, as fabrication conditions are less restrictive without the need to maintain cell viability.
In cellular bioprinting, cells and other biological agents are incorporated into the material during manufacturing to create living tissue constructs.
The printing parameters, biomaterials, and properties of the 3D-printed constructs differ in each category due to the presence or absence of cells and biological substances.
This discussion briefly introduces and examines these two approaches based on the suitable materials for the constructs and the fabrication processes employed. Current limitations, potential solutions, and future directions in bioprinting are also addressed.
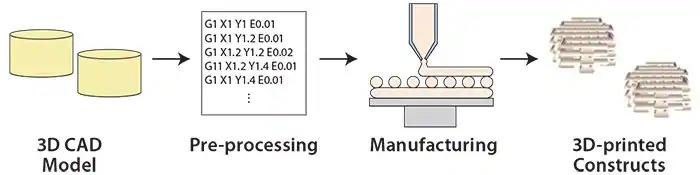
Figure 1. Overview of the 3D printing process. Image Credit: Merck
Manufacturing of acellular scaffolds
An acellular scaffold comprises a porous structure mirroring the mechanical and biochemical properties of the extracellular matrix (ECM). It provides mechanical integrity and serves as a template for cell attachment to stimulate tissue formation.8
Acellular scaffolds must exhibit biocompatible and bioresorbable properties, along with biochemical, biophysical, biomechanical, bioelectrical, and biomagnetic signals.9
Pores in the scaffold play a crucial role, providing space for cell migration and tissue ingrowth, facilitating vasculature formation, and enhancing cell viability.10 Consequently, porosity and porous structures are key features of the scaffold.
The utilization of additive manufacturing (AM) is highly advantageous, allowing precise and repeatable control of scaffold geometry (and, hence, porosity) and enabling the potential assembly of tissue-like spatial complexity.
Various applications of bioprinted acellular scaffolds have been documented, including their use in creating muscular tissues, liver tissues, cartilage, bone, skin, and more.2 The selection of the scaffold's specific material and any potential biological agents is crucial, as they must be carefully chosen to accurately mimic the engineered tissue's properties.
This section will delve into some of the structures that possess robust mechanical strength, typically designed for engineered bone applications.
The materials and additive manufacturing (AM) procedures used for acellular scaffolds related to soft engineered tissues (e.g., skin, liver) mirror those for cell-laden counterparts and are, therefore, detailed in the subsequent section titled "Manufacturing Soft Materials for Cell Encapsulation."
Materials
Four material categories are underscored based on their chemical composition.
The initial category comprises polymers,11 including collagen (Product No. C5483, C7624, H4417, etc.), fibrin (Product No. F5386), alginate, chitosan (Product No. 448869, 448877, 419419, etc.), poly(lactic acid) (PLA) (Product No. 764590, 765112, 764698, etc.), poly(glycolic acid) (PGA) (Product No. 457620 and 46746), polycaprolactone (PCL), and poly(propylene fumarate) (PPF).
These materials can exhibit high bioresorbability and flexibility in terms of chemical composition and processability. However, polymer-based scaffolds demonstrate a swift decline in stiffness post-implantation.
Calcium phosphate (CaPs) (Product No. 21218) based ceramic scaffolds, such as hydroxyapatite (HA) (Product No. 289396, 677418, 693863, etc.) and β-tricalcium phosphate (β-TCP) (Product No. 13204, 21218, and 49963),12 represent another category that has been used in clinical applications.13
CaPs scaffolds, integral to bones, display substantial osteoconductivity and high compressive strength, potentially enhanced by dopant additives like SiO2 (Product No. 805890, 806587, 806765, etc.) or ZnO (Product No. 14439, 96479, etc.). However, their processability is limited, constraining possible geometries.
Metals, typically titanium or stainless steel, are also employed to ensure biocompatibility. While they offer considerable mechanical strength, they are non-biodegradable.13
Composite materials, formed by blending two or more materials, aim to amalgamate the advantages of each component.
A good example of this involves polymer/ceramic composite, like PCL/TCP or PCL/HA, where the ceramic is integrated into the polymer to enhance mechanical integrity and bioactivity.14 These composites have displayed encouraging outcomes for acellular scaffolds, and there are numerous unexplored combinations with significant potential.
Manufacturing process
Various 3D printing and additive manufacturing (AM) methods have been developed and commercialized since the 1980s,15,16 including Stereolithography (SLA), Selective Laser Sintering (SLS), and Fused Deposition Modeling (FDM).
These printing methods are applicable to bioprinting acellular scaffolds due to their reduced need for strict precautions such as speed, temperature, toxicity, and pressure during printing.
SLA involves directing a laser beam horizontally to cure a photosensitive material and create a fixed layer.16 This layer is then vertically moved to enable the formation of the next adjacent layer, as illustrated in Figure 2.
This technology allows high-resolution printing, with a layer thickness as small as 20 μm. The horizontal resolution is determined by the laser's diameter (approximately 250 μm); using Digital Light Projection (DLP) instead of a laser can enhance the resolution to 70 μm.
SLA, however, confines the biochemical composition of constructs to a single, photosensitive material. In contrast, SLS utilizes a high-powered laser (Figure 2) to heat and fuse a powder-based material.
The successive layers are fabricated by rastering the laser over the powder bed. Once each layer is complete, another layer of powder is added on top of the previous one, which will later be sintered by the laser to form the next layer. This is repeated until the entire part is produced.16
Scaffolds produced using Selective Laser Sintering (SLS) exhibit exceptional mechanical strength and intricate shapes. This is attributed to the effective bonding between each layer through sintering, and the presence of unsintered powder that offers support for each subsequent layer.
The resolution and surface finish, however, may vary depending on the characteristics of the powder material used.
FDM 3D printing involves positioning an extruding nozzle to deposit material strands in 3D space. Inside the nozzle, the material is thermally melted, and it solidifies upon cooling as it's deposited, resulting in the creation of each layer (as shown in Figure 2).
Materials used in FDM must possess a molten phase, and certain polymers and composites are thus ideal for use in this process. Due to its strand-based deposition approach, FDM is excellent for producing porous structures. However, manufacturing intricate geometries, such as overhanging layers, can be challenging.
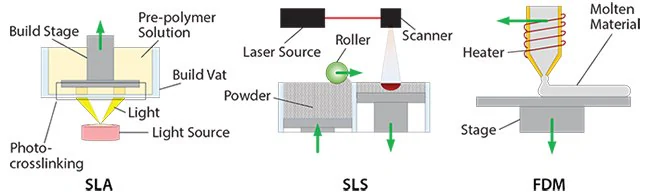
Figure 2. 3D printing processes for the manufacturing of acellular scaffolds. Image Credit: Merck
Manufacturing soft materials for cell encapsulation
While acellular scaffolds offer mechanical support and structural guidance for cell growth, post-processing is necessary for cell seeding and/or biomolecule loading onto the scaffold.
This task is intricate and does not permit controlled attachment or spatial distribution of cells and biomolecules within the scaffold. However, loading is easier to achieve by encapsulating the cells and/or biomolecules directly in the printed material.
The creation of highly intricate tissue constructs is possible through the integration of diverse cell types and growth factors in designed biomimetic patterns.2 Such constructs find applications in various areas, facilitating significant advancements in 3D miniature tissue models for drug delivery testing.
Meeting the demands of these applications requires adherence to sterile conditions, utilization of non-toxic materials, implementation of mild fabrication processes, and maintaining relatively short processing time windows. These factors impact both material selection and the printing process.
Materials
For successful cell encapsulation, the printing material must possess high water content and sufficient porosity to enable cells to receive necessary nutrients and oxygen from the surroundings and expel waste, which is essential for their survival.
The chosen material should be both soft and biodegradable, allowing cells to disperse, move, multiply, and engage with one another.17
Hydrogels, whether natural or synthetic, are the most commonly employed materials in cell encapsulation. Natural hydrogels like gelatin and collagen, sourced from animal or human tissues, exhibit inherent molecular interactions with cells.
Synthetic hydrogels, such as poly(ethylene glycol) or PEG, find widespread use in bioprinting due to the adaptable physical properties they offer. Depending on the gelation principle, hydrogels can be categorized as either physical or chemical hydrogels.15
Physical hydrogels form through alterations in temperature, pH value, or other physical properties and can revert to a liquid state if these conditions return to their initial state. On the other hand, chemical hydrogels are created through irreversible crosslinking via covalent bonds, resulting in a water-insoluble network.
Chemical crosslinking involves combining two mutually reactive chemicals, while photo-crosslinking is accomplished by exposing a solution containing a photosensitive polymer and a photo-initiator to visible or UV light.16
Manufacturing process
The prevalent utilization of inkjet-based bioprinting involves the creation of 3D cell-laden structures by consistently expelling cell-loaded droplets onto a designated platform, facilitated by a thermal or acoustic actuator. The nature of the print head designates the 3D construct be built dot by dot for each layer.
Bioprinters relying on inkjet technology are widely applied due to their rapid printing speed, compatibility with biological components, and cost-effectiveness. The choice of printing material viscosity is crucial to prevent clogging of the print head.
SLA can be employed to fabricate cell-laden structures by incorporating cells into an uncrosslinked pre-polymer substance. The preferred light source for crosslinking in DLP is visible light, considering cell sensitivity to UV exposure and temperature fluctuations.
While SLA offers high resolution, balancing printing quality and overall processing time is essential for optimal cell viability.
SLA requires a larger amount of material compared to other bioprinting methods due to the requirement of filling the vat (Figure 3) with the printing material. This poses a significant drawback, especially when using expensive materials.
Extrusion is a viable alternative for printing hydrogel constructs. In this approach, a chamber is filled with cell-laden biomaterial, and pneumatic or piston-driven extrusion propels the material through the print head. The robotic movement of the print head follows a predetermined path to create cell-laden structures layer by layer.
For physically formed hydrogels, struts are extruded and gelled on stage through changes in pH, temperature, or other physical conditions. Photo-crosslinkable materials can also be utilized, where a layer of pre-polymer solution is extruded and crosslinked upon exposure to light.
Despite precise control over printing speed and extrusion, careful consideration is required to prevent significant shear stress on the material, which may impact cell viability.
Laser-based bioprinting is a widely employed technique that utilizes a laser to transfer cell-laden materials from a source plate to the deposition stage. This transfer is accomplished by coating the source plate with a double layer consisting of a laser-absorbing layer and a donor layer of biomaterial (Figure 3).
A laser pulse focusing on the absorbing layer generates a bubble, propelling a droplet of biomaterial onto the destination stage.
While using a laser instead of a nozzle enables the deposition of highly viscous materials with high accuracy, the generated heat poses challenges to cell viability, meaning that this method is limited when it comes to constructing vertical structures.
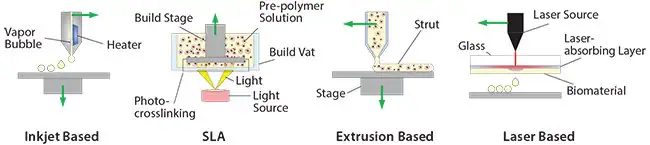
Figure 3. 3D printing process for the manufacturing of soft materials for cell encapsulation. Image Credit: Merck
Current challenges and perspectives
Thus far, this article has provided a concise overview of the state-of-the-art bioprinting processes, focusing on the two primary methods. The initial approach involves utilizing acellular scaffolds to create detailed and consistently replicable implantable templates, fostering cell function and tissue regeneration.
In the second approach, cells are directly encapsulated within the material during the printing process, with the materials characterized by a high water content, hence considered soft in terms of stiffness.
While this discussion intentionally emphasizes stiffer materials in the first approach, it is essential to note that acellular scaffolds based on soft materials can also be accomplished using methods outlined in the earlier section, "Manufacturing Soft Materials for Cell Encapsulation."
Despite exhibiting promising outcomes, bioprinting remains in its early stages, with several challenges demanding attention for progress in the field.
For instance, as mentioned earlier, current 3D bioprinting techniques face limitations in integrating the soft and rigid multifunctional components necessary for tissues and organs due to their inherent heterogeneity in mechanical, physical, chemical, and biological attributes.
In pursuit of this objective, a 3D hybrid bioprinter (Hybprinter) has been introduced, which is capable of seamlessly and swiftly integrating cell-laden soft materials and rigid frame materials using FDM, SLA, and extrusion-based techniques in a controlled and automated manner within a unified platform.18
Leveraging each process allows the manufacturing of constructs featuring both acellular scaffolds and cell-laden hydrogels, marking a stride toward intricate multi-material constructs.
While bioprinting holds promise in tissue engineering, enhancements in the printing processes are imperative. Consideration should be given to increased speed to facilitate the scaling up of acellular scaffolds and enhance cell viability for cell-laden constructs.
Additionally, greater resolution is a must, particularly for the fabrication of diverse composite tissues and vascularized tissues, where vascularization stands out as a pivotal yet formidable challenge in tissue engineering.19
Tissues of this nature comprise a highly intricate vascular network, ranging from millimeter-sized vessels to micrometer-scale capillaries. Replicating such a network poses a significant challenge, and thus far, efforts have primarily focused on allowing sufficient space in the porous scaffold for vascular tissues to develop spontaneously.
A 3D printing method, like two-photon polymerization, enables the production of components within the micron-to-millimeter size range and is hence regarded as a promising bioprinting technique for creating vascularized tissue structures.
Research should focus on the development of new materials with enhanced biological properties suitable for bioprinting. Attention should also be directed towards leveraging current or future technologies to enhance the assembly of existing biomaterials, aiming to better imitate the complexity of the ECM or a combination of both approaches.
While available materials for cell-laden tissue constructs dedicated to bioprinting are currently limited, advancements in imaging capabilities and a deeper understanding of tissue complexity and developmental biology will contribute to the evolution of new materials and bioprinting technologies.
The extent to which achieving biomimetic complexity in both the chemistry and physical structure of bioprinted constructs is necessary for improved healing, and the restoration of lost functions is still a matter of ongoing research and investigation.
An increasing number of applications in additive manufacturing (AM) are emerging, with bioprinting standing out as one of the most promising and challenging manufacturing processes due to its potential impact on global healthcare issues such as aging, organ transplantation, cancer therapy, and personalized and precision medicine.
Looking forward, bioprinting has the potential to serve as both a source of miniature disease and toxicology models for the pharmaceutical industry and as a provider of life-sized tissue/organ replacements for clinical treatments.
Acknowledgments
The financial support from various agencies is gratefully acknowledged: NIH R01AR057837 (NIAMS), NIH R01DE021468 (NIDCR), DOD W911NF-14-1-0545 (DURIP), DOD W81XWH-10-1-0966 (PRORP), and Stanford Coulter Translational Seed Grant.
References
- Stock UA, Vacanti JP. 2001. Tissue Engineering: Current State and Prospects. Annu. Rev. Med.. 52(1):443-451. https://doi.org/10.1146/annurev.med.52.1.443
- Langer R, Vacanti J. 1993. Tissue engineering. Science. 260(5110):920-926. https://doi.org/10.1126/science.8493529
- Griffith LG. 2002. Tissue Engineering--Current Challenges and Expanding Opportunities. 295(5557):1009-1014. https://doi.org/10.1126/science.1069210
- Dababneh AB, Ozbolat IT. 2014. Bioprinting Technology: A Current State-of-the-Art Review. 136(6): https://doi.org/10.1115/1.4028512
- Smith IO, Liu XH, Smith LA, Ma PX. 2009. Nanostructured polymer scaffolds for tissue engineering and regenerative medicine. WIREs Nanomed Nanobiotechnol. 1(2):226-236. https://doi.org/10.1002/wnan.26
- Mironov V, Reis N, Derby B. 2006. Review: Bioprinting: A Beginning. Tissue Engineering. 12(4):631-634. https://doi.org/10.1089/ten.2006.12.631
- Sears NA, Seshadri DR, Dhavalikar PS, Cosgriff-Hernandez E. 2016. A Review of Three-Dimensional Printing in Tissue Engineering. Tissue Engineering Part B: Reviews. 22(4):298-310. https://doi.org/10.1089/ten.teb.2015.0464
- Bose S, Vahabzadeh S, Bandyopadhyay A. 2013. Bone tissue engineering using 3D printing. Materials Today. 16(12):496-504. https://doi.org/10.1016/j.mattod.2013.11.017
- Hutmacher DW. 2000. Scaffolds in tissue engineering bone and cartilage. Biomaterials. 21(24):2529-2543. https://doi.org/10.1016/s0142-9612(00)00121-6
- Bose S, Roy M, Bandyopadhyay A. 2012. Recent advances in bone tissue engineering scaffolds. Trends in Biotechnology. 30(10):546-554. https://doi.org/10.1016/j.tibtech.2012.07.005
- Liu, X. H.; Ma, P. X.. 2004. Ann.Biomed. Eng. , 32(3), 477–86. doi: 10.1023/B:ABME.0000017544.360 01.8e. PubMed PMID: WOS:000222465100019..
- Sweet L, Kang Y, Czisch C, Witek L, Shi Y, Smay J, Plant GW, Yang Y. Geometrical versus Random ?-TCP Scaffolds: Exploring the Effects on Schwann Cell Growth and Behavior. PLoS ONE. 10(10):e0139820. https://doi.org/10.1371/journal.pone.0139820
- Lichte P, Pape H, Pufe T, Kobbe P, Fischer H. 2011. Scaffolds for bone healing: Concepts, materials and evidence. Injury. 42(6):569-573. https://doi.org/10.1016/j.injury.2011.03.033
- Lu L, Zhang Q, Wootton D, Chiou R, Li D, Lu B, Lelkes P, Zhou J. 2012. Biocompatibility and biodegradation studies of PCL/?-TCP bone tissue scaffold fabricated by structural porogen method. J Mater Sci: Mater Med. 23(9):2217-2226. https://doi.org/10.1007/s10856-012-4695-2
- Hoffman AS. 2002. Hydrogels for biomedical applications. Advanced Drug Delivery Reviews. 54(1):3-12. https://doi.org/10.1016/s0169-409x(01)00239-3
- Elomaa L, Pan C, Shanjani Y, Malkovskiy A, Seppälä JV, Yang Y. Three-dimensional fabrication of cell-laden biodegradable poly(ethylene glycol-co-depsipeptide) hydrogels by visible light stereolithography. J. Mater. Chem. B. 3(42):8348-8358. https://doi.org/10.1039/c5tb01468a
- Elomaa L, Kang Y, Seppälä JV, Yang Y. 2014. Biodegradable photocrosslinkable poly(depsipeptide-co-?-caprolactone) for tissue engineering: Synthesis, characterization, and In vitro evaluation. J. Polym. Sci. Part A: Polym. Chem.. 52(23):3307-3315. https://doi.org/10.1002/pola.27400
- Shanjani Y, Pan CC, Elomaa L, Yang Y. A novel bioprinting method and system for forming hybrid tissue engineering constructs. Biofabrication. 7(4):045008. https://doi.org/10.1088/1758-5090/7/4/045008
- Mercado-Pagán ÁE, Stahl AM, Shanjani Y, Yang Y. 2015. Vascularization in Bone Tissue Engineering Constructs. Ann Biomed Eng. 43(3):718-729. https://doi.org/10.1007/s10439-015-1253-3
- Weiß T, Berg A, Fiedler S, Hildebrand G, Schade R, Schnabelrauch M, Liefeith K. 2009. Two-Photon Polymerization for Microfabrication of Three-Dimensional Scaffolds for Tissue Engineering Application.140-142. https://doi.org/10.1007/978-3-642-03900-3_41
About Merck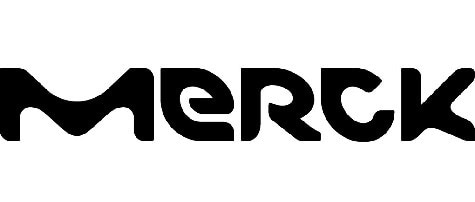
Our pursuit is progress for people everywhere. That's why we take a closer look at things, ask questions, and think ahead.
We've been around for more than 350 years, yet our majority owners are still the descendants of Friedrich Jacob Merck, the man who founded our company in Darmstadt, Germany in 1668.
From advancing gene-editing technologies and discovering unique ways to treat the most challenging diseases to enabling the intelligence of devices – the company is everywhere.
We are Merck. The only exceptions are the United States and Canada. Here we operate as EMD Serono in the Biopharma business, as MilliporeSigma in the Life Science business, and as EMD Performance Materials in the materials business.
Our life science business
We provide infinite solutions to solve the toughest problems in life science in collaboration with the global scientific community. Our tools, services, and digital platforms empower scientists and engineers at every stage, helping deliver breakthrough therapies more quickly.
Focus areas
With our three business units, we are a leading worldwide supplier of tools, high-grade chemicals, and equipment for academic labs, biotech, and biopharmaceutical manufacturers, as well as the industrial sector.
- Research Solutions provides our academic customers with the chemicals and tools needed to make scientific discovery easier and faster.
- Process Solutions provides drug manufacturers with process development expertise and technologies, such as continuous bioprocessing.
- Applied Solutions offers both testing kits and services to ensure that our food is safe to eat and our water is clean to drink.
Sponsored Content Policy: News-Medical.net publishes articles and related content that may be derived from sources where we have existing commercial relationships, provided such content adds value to the core editorial ethos of News-Medical.Net which is to educate and inform site visitors interested in medical research, science, medical devices and treatments.