Widespread neurological conditions, including Alzheimer’s disease, Parkinson’s disease, epilepsy, multiple sclerosis, stroke, and brain tumors, are among the top causes of disability and death around the world and bear a significant clinical, social, and economic burden.
Robust studies have unveiled complicated interactions among the molecular and cellular parts of nervous tissue, cerebral vasculature, and the immune system implicated in the genesis, progression, and maintenance of central nervous system (CNS) conditions.
These processes' versatile character and interaction demand system approaches to research them inside an intact organism. Explorations are typically carried out in lab mice and rats due to both genetic and biological similarities to humans and the considerable variety of neurological disease conditions that can be triggered via experimental manipulation, genetic engineering, and changing lifestyle factors such as diet and environment.
New therapeutic techniques, including immuno-, gene and cell therapy, brain-machine interfaces, and precise drug delivery approaches designed to reduce or cure neurological conditions, for which frequently few effective approaches exist, necessitate comprehensive preclinical evaluation in animal models before their translation to patients. Thus, approaches that non-invasively enable the visualization of cellular and molecular processes of the CNS in small animals are extremely sought-after.
Bruker provides a portfolio of preclinical imaging tools, including magnetic resonance imaging (MRI), positron emission tomography (PET), and single photon emission computed tomography (SPECT), suitable for cellular and molecular imaging use cases.
Among them, MRI has found significant application in examining CNS pathology, delivering data about changes in the anatomy, organization, and circuitry of the brain and spinal cord, and producing quantitative MRI measures of microstructure and tissue composition alongside metabolic and functional processes.
Bruker’s dynamic MRI tool design includes BioSpec Maxwell tools beginning with 3 Tesla, classic MRIs, and ultra-high field MRIs like the BioSpec 18 Tesla. Tools are fitted with cutting-edge hardware parts from high-performance gradients to the electronic architecture to radiofrequency coils, committed to imaging the brain and spinal cord of both mice and rats alongside other model organisms, that collectively enable data collection both quickly and at a high quality, covering a wide variety of use cases.
Bruker’s ParaVision software is accompanied by protocols that have been pre-optimized for small rodent species and associated use cases. It comprises sophisticated imaging viewing, reconstruction, and analysis options and a software framework for developing individual MR methods.
Recent progress in disciplines, including chemistry, molecular and cell biology, and nanotechnology, has increased MRI’s capabilities to encompass the identification of pathological changes in tissue via instruments that visualize both cellular and molecular processes.
Numerous novel imaging probe reporter genes and cell-labeling approaches form the foundations for gaining an unparalleled understanding of the pathomechanisms behind neurological conditions. In this paper, Bruker illustrates examples from academic work on the use of molecular and cellular MRI in models of neurological conditions alongside its use for evaluating both gene and cell therapies.
Mapping molecular processes and gene expression
Targeted MRI probes are developed to visualize specific pathophysiological molecular processes. They are typically composed of contrast agents like iron oxide nanoparticles, paramagnetic compounds (e.g., Gadolinium), chemical exchange saturation transfer (CEST)- and paramagnetic CEST (PARACEST)- agents, or perfluorocarbons (19F) and a targeting moiety like an antibody, peptide, oligonucleotide or enzyme substrate (Fig. 1).
Microsized matrix-based magnetic particles (M3P) were utilized for detecting brain inflammation.1 M3Ps were fabricated via self-assembly of catechol-coated magnetite nanocrystals (Fig. 2A). The M3Ps have a high payload of superparamagnetic material and demonstrate biocompatibility and water dispersibility.
M3Ps conjugated antibodies against the vascular cell adhesion molecule 1 (VCAM-1) to indicate endothelial activation. T2*-weighted MRI demonstrated local accumulation of VCAM-1-targeted M3P in the striatum following lipopolysaccharide injection, which led to focal neuroinflammation. M3P was additionally utilized to identify the inflammatory response to focal cerebral ischemia inside the mouse brain.
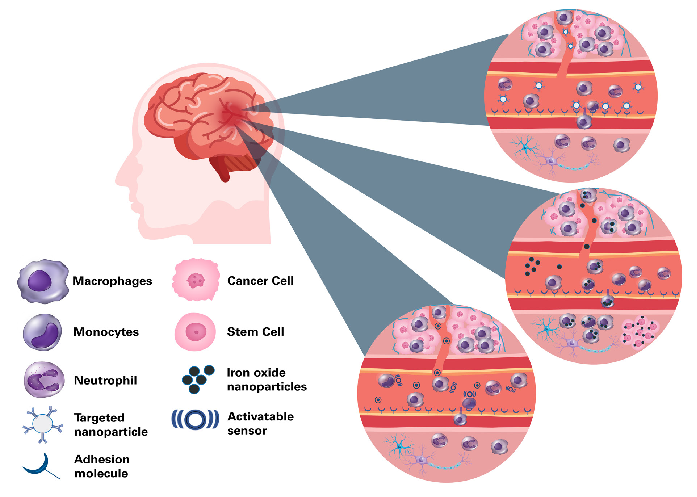
Figure 1. Approaches for cellular and molecular imaging of brain tumors. Top: Targeted MRI probes bind to receptors that are highly expressed in tumors, for example vascular adhesion molecules. Middle: Cell labeling and reporter genes can track the dynamics of immune cell trafficking and the fate of cell-based therapies. Cells can either be labeled with an MRI contrast agent, often an iron oxide nanoparticle, or be transfected with a plasmid containing a reporter gene. The gene product in the cells gives rise to a detectable MRI contrast. Labeled cells are either transplanted locally (e.g. stem cells) or are injected systematically (e.g. monocytes), where they migrate to their target site. Alternatively, nanoparticles are injected intravascularly where they are taken up by phagocytosing cells like macrophages. Bottom: Injected activatable sensors report enzyme activity, thus providing highly specific molecular markers of the tumor pathology. Image Credit: Bruker BioSpin - NMR, EPR and Imaging
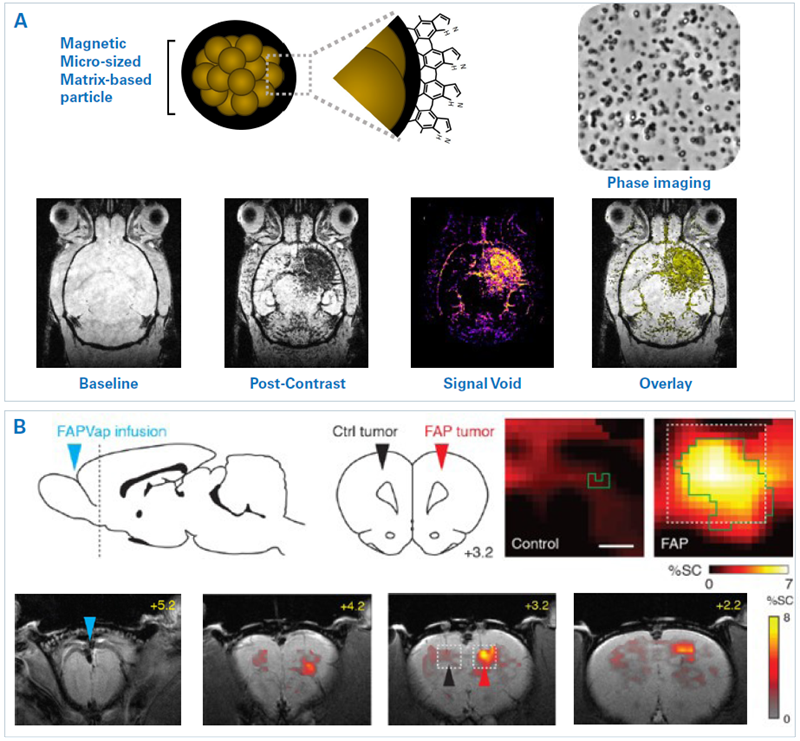
Figure 2. Examples of molecular MRI. A) Immuno-MRI with microsized matrix-based magnetic particles (M3P). Top: Representation of the structure and surface of MP3 and phase contrast microscopy. Bottom: T2*-weighted images of a mouse brain are depicted at baseline and 10 minutes after injection of M3P conjugated with monoclonal antibodies against VCAM-1. The mouse had been injected with lipopolysaccharide into the right striatum 24 h prior MRI. The resulting signal void and overlay are also shown. B) Hemodynamic molecular MRI of tumor-associated enzyme activity. Top left: An FAP-activatable probe was infused into the subarachnoid space caudal to the olfactory bulb and rostral to the tumors (blue arrowhead). FAP-expressing (red arrowhead) and control (black arrowhead) tumors were inoculated into the dorsal cortex (dashed line) in rats. Bottom: T2*-weighted MRI reveal signal change (color) following FAP-sensitive vasoprobe infusion in the presence of control (black arrowhead) and FAP-expressing (red arrowhead) xenografts overlayed on an anatomical image. Scale bar = 4 mm. Top right: Zoom-ins on tumor regions (dotted boxes). Scale bar = 0.5 mm. Image Credit: Images were taken from Martinez de Lizarrondo et al. 20221; Desai et al. 20212 with modifications
In another method, an activatable probe was developed and utilized for detecting fibroblast activation protein (FAP) activity in brain tumors.2 FAP is highly expressed by many tumor varieties, such as glioblastomas. Sensors are developed from peptides that are fused to an FAP-labile blocking domain. When the FAP uncages the peptide, it generates vasodilation, which is detectable by MRI.
The potential of identifying FAP-expressing tumors in vivo was demonstrated (Fig. 2B). FAP-expressing and control tumors were inoculated into the dorsal cortex in rats. The FAP-activatable probe was infused into the subarachnoid space caudal to the olfactory bulb and rostral to the tumors. T2*-weighted MRI demonstrated probe activation at the FAP-expressing tumor, highlighting the method’s specificity.
Reporter gene assays are built to study the expression of specific genes (e.g., oncogenes, cytokine—or chemokine-related genes). Reporter genes are transfected into cells or carried by viral vectors to specific tissues (Fig. 1). The gene of interest and the reporter protein are motivated by the same promoter. The resultant reporter proteins generate an identifiable MRI contrast when both genes are expressed.
Common MRI reporters include iron chelating and storing proteins or enzymes (such as tyrosinase and ferritin) that heighten the intracellular concentration of paramagnetic iron, thus diminishing rates of relaxation and generating contrast in T1- and T2-weighted MR images.3 Alternative methods seek to raise intracellular protein concentration or alter cellular water diffusivity.4,5
A lysine-rich protein (LRP) reporter gene has been developed using the reporter MRI method.4 The LRP reported was expressed in glioma cells that were inoculated into mice brains (Fig. 3A). Glioma cells transfected with an empty construct were the control. CEST-MR images were obtained prior to and following oncolytic herpes simplex virus (oHSV) therapy. A considerable increase in the tumor amide-CEST contrast was acquired for LRP-expressing tumors in comparison to the control which was intensified by the oHSV treatment.
A different approach utilized aquaporin 1 (AQP1) as a novel genetically encoded reporter.5 AQP1 expression changes tissue water diffusivity in a way that is detectable with diffusion-weighted MRI (Fig. 3B).
Tumor cells expressing AQP1 or green fluorescent protein as controls were inoculated into a mouse's right and left striatum. Reporter gene expression was regulated by doxycycline administration. Diffusion MRI demonstrated a considerable decrease in signal in the AQP1 tumor following treatment with doxycycline.
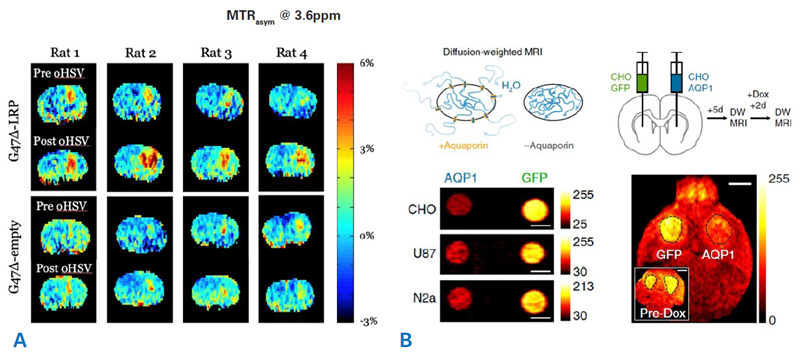
Figure 3. Examples molecular MRI using reporter gene approaches. A) CEST-MRI imaging of oncolytic virus therapy in brain tumors using LRP reporter. Shown are amide proton CEST amplitude maps of eight rats before and after oncolytic herpes simplex virus (oHSV) therapy. Animals were inoculated with tumor cells that were transfected to express either LRP (G47Δ-LRP) or received a control construct (G47Δ-empty). The oncolytic virus therapy leads to an increased LRP signal within the tumors. Courtesy: Christian T. Farrar, Department of Radiology, Athinoula A. Martinos Center for Biomedical Imaging, Massachusetts General Hospital and Harvard Medical School, USA. B) Aquaporin 1 (AQP1) as a new genetically encoded reporter for diffusion weighted MRI. Top: Illustration showing the change in water diffusion when a cell expresses aquaporin receptors. Bottom left: Diffusion-weighted images of CHO, U87, and Neuro 2a cell expressing either AQP1 or green fluorescent protein. Scale bars = 3 mm. Bottom right: Tumor cells expressing either AQP1 or green fluorescent protein under the CHO promotor were inoculated into the right and left striatum of a mouse. Diffusion-weighted MRI was performed before and after doxycycline (Dox) administration. Representative diffusion-weighted image of a coronal brain slice with bilateral tumor xenografts, 48 h after doxycycline administration. Inset shows a diffusion-weighted image of the same mouse acquired before doxycycline injection. Scale bar = 2 mm. Image Credit: Images were taken from Mukherjee et al. 20165 with modifications
Tracking cell trafficking and cell therapies
It is possible to use MRI to monitor cell populations implicated in the development of neurological conditions (e.g., tumors, immune cells) and their repair (e.g., neuronal stem cells, oligodendrocyte precursors, or macrophages). Cells can be labeled by contrast agents (iron oxide nanoparticles, 19F compounds, etc.) or reporter genes (Fig. 1).
Utilizing reporter gene methods has certain advantages. It enables cell labeling and reporting on cellular functional states when specific genes are expressed. Cells can be labeled or transfected in vitro and then implanted or injected into an animal.
Contrast agents may also be systemically injected, in which case they are absorbed by phagocytotic cells. However, a key issue is the presence of blood-brain or blood-spinal cord barriers that can hamper the delivery of systemically administered cells to their target area within the CNS. Cell delivery may be used alongside methods to disrupt or overcome barriers like MRI-guided focused ultrasound.
In MRI cell tracking, signals are typically identified from bulk cells within tissue. However, real-time single-cell tracking in the mouse brain has also been shown.6 T2*-weighted images of a naïve mouse obtained with 20 timeframes (from 8 to 164 minutes) following intravenous injection of iron oxide nanoparticles demonstrate hypointense spots, representing mononuclear phagocytes that have absorbed the injected nanoparticles (Fig. 4A).
Moreover, time-lapse MRI was used on mice with experimental autoimmune encephalomyelitis (EAE) and control mice following the injection of monocytes exogenously labeled with iron oxide nanoparticles. Labeled monocytes move to the inflammatory lesion in the brain and the lesion in EAE mice. The quantity of counted hypointense spots was considerably elevated in naïve as opposed to EAE mice, at the pre-symptomatic and symptomatic phases alike, demonstrating adhesion of patrolling monocytes to the vasculature at inflammation sites in EAE mice.
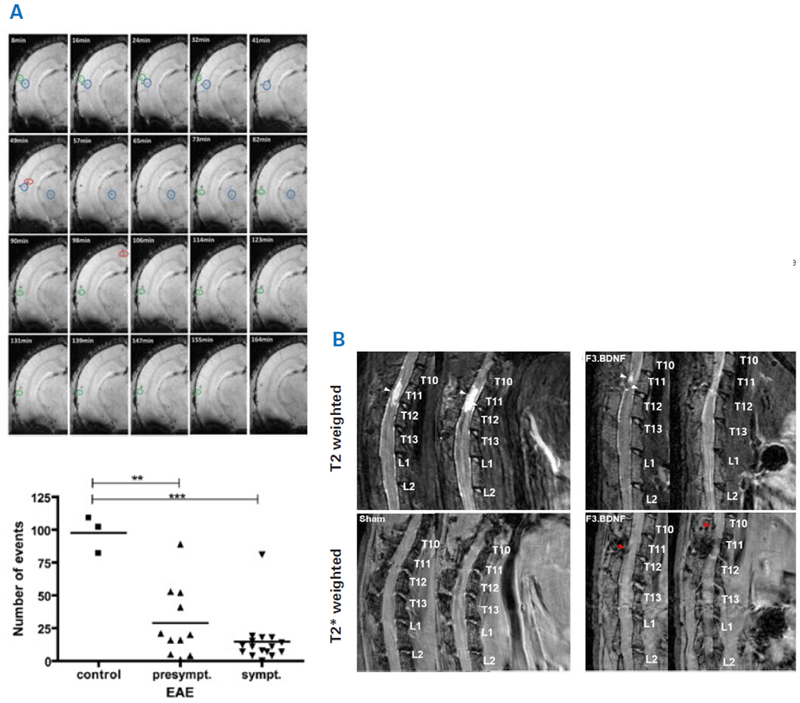
Figure 4. Examples for using MRI for examining cell therapy. A) Top: Time-lapse MRI for tracking inflammatory cells. Shown are 20 time frames (8, 16,…until 164 min) of one coronal T2*-weighted image from a brain of naïve mouse after intravenous injection of iron oxide nanoparticles. Hypointense spots represent labelled immune cells. Bottom: Cell trafficking was assessed in EAE and control mice after injection of iron oxide nanoparticle labelled monocytes. The number of counted hypointense spots (overall events) were significantly higher in naïve as compared to EAE mice, both at the pre-symptomatic and symptomatic stage. **p < 0.01 and ***p < 0.001. B) Detection of Feridex®-labeled human neural stem cells overexpressing brain-derived neurotrophic factor (F3.BDNF) in a rat contusion model of spinal cord injury. T2-weighted and T2*-weighted MRI images at 72 days after contusive spinal cord injury and infusion of either F3.BDNF cells or medium as control. The white arrowheads indicate the lesion, while the red arrowheads indicate labeled F3.BDNF cells. There is a marked reduction in the hyperintense lesion between the F3.BDNF group and control. Image Credit: Images were taken from Masthoff et al. 20186; Chang et al. 20217 with modifications.
It is also possible to use MRI to track cell and tissue transplants seeking to replace and repair damaged cells and/or tissue and to enhance endogenous restorative mechanisms that aim to repair or regenerate damaged nervous tissue. It enables the trafficking of cells to the target site and the evaluation of engraftment and long-term viability.
The transplantation of human neural stem cells overexpressing brain-derived neurotrophic factor (F3.BDNF) into a rat spinal cord was investigated as a potential therapy for a contusion injury.7 F3.BDNF cells were labeled with Feridex® before transplantation. T2-weighted imaging was utilized to mark the development of the lesion, and T2*-weighted imaging was utilized to detect transplanted F3.BDNF cells (Fig. 4B).
Labeled F3.BDNF cells were found to traffic to the lesion site, which was associated with a marked reduction in the hyperintense lesion compared to the control. Moreover, immunohistochemistry demonstrated that the transplanted F3.BDNF differentiated into neurons, oligodendrocytes, and astrocytes in the injured spinal cord. Cell transplantation reduced inflammatory response at the lesion site and resulted in enhanced functional recovery in the injured rats.
MRI and magnetic resonance spectroscopy (MRS) are also important instruments for assessing the behavior of incorporated cells and tissue graft in the host tissue as well as functional tissue changes that stem from therapy like assessing gross structural changes (e.g. reduction in lesion extent), tissue remodeling (e.g. remyelination, angiogenesis, changes in connectivity) and functional tissue changes (e.g. neuroplasticity, neurotransmitter concentration).
Theranostics and evaluation of gene therapies
MRI probes can be used as theranostics when they consist of therapeutic agents and signal moiety. MRI can also track the therapeutic agents' delivery and/or release at the target site. For instance, 19F MRI was utilized to image Siponimod, a drug utilized in multiple sclerosis to reduce the infiltration of inflammatory lymphocytes into the CNS.8
Following oral administration in mice, the drug was identified with 19F MRI in vivo in the stomach and over time in the liver as well, showcasing the drug's absorption from the stomach (Fig. 5A). Ex vivo 3D ultra-short echo-time (UTE) MR images of the brain indicated that the drug passes over the blood-brain barrier and builds up in brain areas at various concentrations.
Gene therapy delivers functional genetic material to cells in the CNS to improve neurological conditions linked to missing or faulty genes and treat brain tumors and brain and spinal cord injuries. Faulty genes can be rectified by introducing a functional copy of the gene by silencing a mutant allele utilizing ribonucleic acid (RNA) interference, introducing a disease-modifying gene, or utilizing gene-editing technology (e.g., CRISPR/Cas).
MRI can be useful for guiding the focal administration of gene material. Once a copy of a therapeutic gene has been delivered to affected cells in the CNS, the product encoded by that gene (i.e., its messenger RNA and/or proteins) will be generated inside the cells using the cell's transcriptional and translational machinery, providing a potentially life-long therapeutic effect.
When delivered, the stably expressed transgene(s) can be observed via MRI utilizing reporter gene approaches or probes. MRI and MRS probe tissue properties and provide an early evaluation of therapeutic efficiency and longitudinally track therapeutic effects. For instance, serial MRI and MRS were utilized to track adeno-associated virus-mediated gene delivery therapy in a mouse model of Canavan disease.9
In aspartoacylase (ASPA)- deficient lacZ-knock-in (AKO) mice, ASPA deficiency induces an accumulation of N-acetyl-L-aspartate (NAA) and, as a result, leukodystrophy. Serial T2-weighted MR imaging showed progressive hyperintensities in the thalamus in AKO mice, resulting from spongiform vacuolization in disease progression. (Fig. 5B).
Adeno-associated virus-mediated gene delivery in AKO mice at 22 weeks old diminished brain NAA levels and improved thalamic hyperintensities four weeks after injection. Other brain metabolites were found to normalize to control levels nine weeks after therapy. Gene therapy also reduced astrogliosis, demyelination, and locomotor impairment observed in untreated AKO mice.
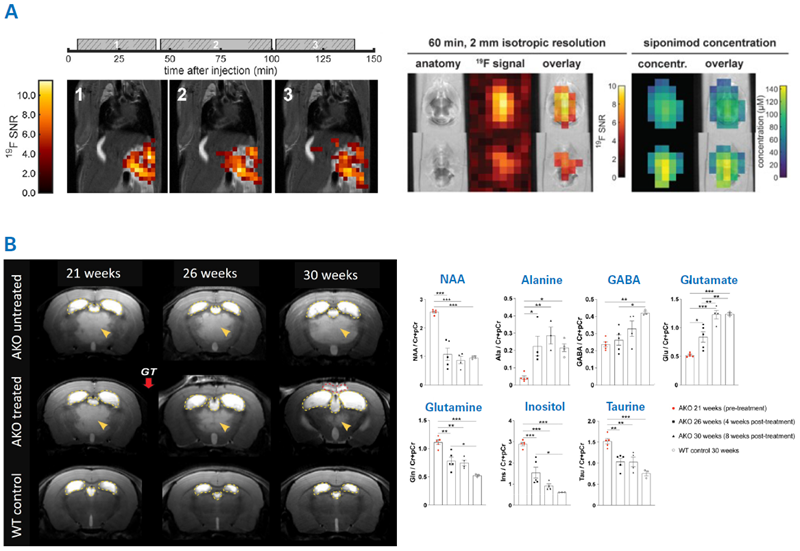
Figure 5. Examples for using MRI for examining theranostic and gene therapy. A) Theranostic MRI of the multiple sclerosis drug Siponimod. Left: 2D 19F UTE images overlaid on anatomical 1H images. Each image corresponds to the average of 3 × 10 min 19F acquisition acquired at different time intervals after oral administration of Siponimod (gray boxes). Middle and right: 3D UTE 19F MRI in ex vivo brain tissue and Siponimod concentration estimates. B) MRI therapy monitoring of adeno-associated virus-mediated gene delivery to a Canavan disease mouse model. Aspartoacylase (ASPA)-deficient lacZ-knock-in (AKO) mice replicate Canavan disease-like pathology. Left: Serial T2-weighted MR imaging revealed hyperintensities in the thalamus in AKO mice (yellow arrowheads) resulting from spongiform vacuolization. Yellow dashed lines outline the lateral and third ventricles. Adeno-associated virus-mediated gene therapy in AKO mice (AKO treated) at 22 weeks of age resulted in reduced vacuolization. Right: Serial MRS was used to assess changes in brain metabolites in response to the gene therapy. Gene therapy reduced NAA levels in AKO mice and normalized metabolite levels at 30 weeks of age. Image Credit: Images were taken from Starke et al. 20238; Fröhlich et al. 20229 with modifications.
Conclusion
Dedicated MRI imaging probes, cell labeling, and reporter gene strategies have enabled MRI to evolve from a modality able to report structural, functional, and metabolic tissue changes to an instrument that can directly report cellular and molecular pathophysiological processes in an intact animal.
Cellular and molecular MRI has been especially helpful for characterizing the tumor microenvironment and evaluating novel immune, gene, and cell therapies for treating neurological conditions by providing direct readouts of therapy delivery and tissue response.
References and further reading
- De Lizarrondo, S.M. et al. (2022). Tracking the immune response by MRI using biodegradable and ultrasensitive microprobes, Science Advances, 8(28). https://doi.org/10.1126/sciadv.abm3596.
- Desai, M. et al. (2021). Hemodynamic molecular imaging of tumor-associated enzyme activity in the living brain, eLife, 10. https://doi.org/10.7554/elife.70237.
- Cai, A. et al. (2021). Longitudinal neural connection detection using a ferritin‐encoding adeno‐associated virus vector and in vivo MRI method, Human Brain Mapping, 42(15), pp. 5010–5022. https://doi.org/10.1002/hbm.25596.
- Perlman, O. et al. (2020). Redesigned reporter gene for improved proton exchange-based molecular MRI contrast, Scientific Reports, 10(1). https://doi.org/10.1038/s41598-020-77576-z.
- Mukherjee, A. et al. (2016). Non-invasive imaging using reporter genes altering cellular water permeability, Nature Communications, 7(1). https://doi.org/10.1038/ncomms13891.
- Masthoff, M. et al. (2018b). Temporal window for detection of inflammatory disease using dynamic cell tracking with time-lapse MRI, Scientific Reports, 8(1). https://doi.org/10.1038/s41598-018-27879-z.
- Chang, D.-J. et al. (2021). Therapeutic effect of BDNF-Overexpressing human neural stem cells (F3.BDNF) in a contusion model of spinal cord injury in rats, International Journal of Molecular Sciences, 22(13), p. 6970. https://doi.org/10.3390/ijms22136970.
- Starke, L., et al. (2023). First in vivo fluorine-19 magnetic resonance imaging of the multiple sclerosis drug siponimod. Theranostics, 13(4), pp.1217–1234. https://doi.org/10.7150/thno.77041.
- Fröhlich, D. et al. (2022). Dual-function AAV gene therapy reverses late-stage Canavan disease pathology in mice, Frontiers in Molecular Neuroscience, 15. https://doi.org/10.3389/fnmol.2022.1061257.
About Bruker BioSpin - NMR, EPR and Imaging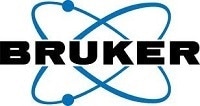
Welcome to Bruker BioSpin and the world's most comprehensive range of NMR and EPR spectroscopy and preclinical imaging research tools. The Bruker BioSpin Group of companies develop, manufacture and supply technology to research establishments, commercial enterprises and multi-national corporations across countless industries and fields of expertise.
Bruker BioSpin is continuing to revolutionize the design, manufacture and distribution of life science, preclinical, process control and analytical research tools based on magnetic resonance and multimodal imaging technologies. Bruker BioSpin is the worldwide technology and market leader in magnetic resonance technologies (NMR, EPR) and offers the largest portfolio of imaging modalities for preclinical and industrial research under a single brand.
Sponsored Content Policy: News-Medical.net publishes articles and related content that may be derived from sources where we have existing commercial relationships, provided such content adds value to the core editorial ethos of News-Medical.Net which is to educate and inform site visitors interested in medical research, science, medical devices and treatments.